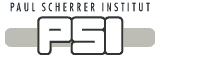 |
THE MULTILEVEL PROTECTION SYSTEM
FOR THE VACUUM CHAMBERS OF THE
HIGH-INTENSITY 590 MEV PROTON BEAM LINES
|
|
Important facts for safely operating the PSI high intensity megawatt proton beam lines and neutron spallation source.
Printable pdf version of this page
(from PSI Scientific and Technical Report 2003, Large Research Facilities, Volume VI, p45-48)
Abstract: A multilevel protection system developed by many specialists and in operation since decades has constantly been upgraded
to the needs of the today's 590 MeV high-intensity proton beam lines (power > 1 megawatt), which is very demanding on the reliability of
diagnostic elements and electronic equipment in order to avoid long and costly shut-downs caused by a damaged vacuum
chamber in a highly radioactive environment.
|
The range of 590 MeV protons in steel is about 28 cm. Therefore, the PSI high intensity megawatt proton beam with its
small diameter and with more than one megawatt of power acts like a welding torch when it hits
the steel walls of a vacuum chamber. At the Targets M and E for example, the diameter of the megawatt proton beam is as
small as 4 mm (sigma-projected = 1mm). For this case, the time to heat up steel to its melting
point as a function of proton beam intensity is given in Fig. 1 (17 kB).
For a 2 mA proton beam, the machine interlock system has to switch-off the megawatt proton beam in less than about 5 ms
to avoid a damaged vacuum chamber or seal where the megawatt proton beam diameter is small. It should be pointed out here,
that a hole in one of the vacuum chambers in the Target E region could cause a shutdown of up to one year duration.
In order to protect the proton-channel vacuum-chamber system from being damaged by the megawatt proton
beam, five different classes of devices or services are installed:
A simultaneous effectiveness of 2-3 of these functions at most locations along the megawatt proton beam line
is desired for redundancy. [Triple safety is an old wisdom. Already the wise king Salomon knew 3000 years ago:
A cord of three strands is not quickly broken. (Ecclesiastes 4,12; The Holy Bible, New
International Version)]
1. Watchdog for magnet currents remaining inside limits.
The actual values of the currents of all bending-magnets along the 590 MeV high intensity megawatt proton beam line
between the ring extraction and the SINQ-target are permanently monitored by their local
COMBI-controllers, when a magnet's value exceeds it's individually programmed upper or lower limit,
then the proton beam is switched off via the machine interlock system. If this function would be absent,
then the proton beam could easily drill a hole into the vacuum chamber of this magnet, because the
produced spill by a wrongly steered proton beam is usually well shielded by the iron yoke and therefore the spill's
intensity may be too weak to be monitored as dangerous by the nearby ionisation chamber. The widths
of the windows created by the upper and lower limits for each magnet have to be wide enough
to allow set-up and tuning sessions with a certain variability of the proton beam energy and direction at
the accelerator exit.
Table 1:
Lower and upper limits
for the back-readings of
the actual values of the
p-channel bending-magnets.
|
Bend's name |
Lower lim. |
Upper lim. |
AHA |
8.40 V |
9.99 V |
AHB |
7.17 V |
8.54 V |
AHC |
7.90 V |
8.20 V |
AHD1 |
8.30 V |
8.70 V |
AHD2 |
8.80 V |
9.40 V |
AHL |
-8.56 V |
-7.75 V |
AHM |
7.94 V |
8.94 V |
AHN |
7.30 V |
8.32 V |
AHO |
7.90 V |
8.90 V |
|
The back-readings (measured in volts) of the lower and upper limits of the 9 bending-magnet's
currents vary from magnet to magnet and are shown in table 1. Each COMBI-controller is also
permanently comparing (with a hardware-comparator) the magnet's set-point value with its actual value
and generating an interlock if it is incorrect.
2. Monitoring the proton beam losses with ionisation chambers.
The backbone of the high intensity megawatt proton beam line's protection system is an array of 29 ionisation chambers
[IC] lined up, at an average distance of about 4 meters, along the proton beam line and close
to the beam tube. They are arranged in 4 interlock groups:
from: |
to: |
# of ICs |
Accelerator exit |
Target M |
9 |
Target M |
Target E |
6 |
Target E |
Beam-dump |
5 |
AHL-bend |
SINQ-target |
9 |
As an example Fig. 2 (12 kB) shows
the operator console's display screen of the 9 ionisation chamber readings for the proton beam line
to SINQ (for the position of MHIxx along the proton beam line see
Fig. 3 {43 kB}) for a proton beam intensity of 1.8 mA (power > 1 megawatt)
extracted from the high intensity ring cyclotron.
The ionisation chambers exist in three shapes (square box, cylinder or ring) and have an active volume of
about one litre each. The applied voltage over the plates inside is 200 volts and the filling gas is
normal air. They are manufactured at PSI and consist of metal and ceramic insulator material only.
There are two basically different programs residing in the ionisation chamber electronics (LOGCAM2 CAMAC
unit developed at PSI) to detect measured values exceeding limits and therefore triggering a
machine interlock in as short a time as 5 ms:
- Program A: see Fig. 4A (11 kB)
As soon as a measured IC current exceeds a hardware (HW) limit, a machine
interlock signal is produced by the electronics. At low proton beam intensities this limit may be to far
away to detect a miss-steered proton beam and to turn off the beam before a vacuum leak is created.
Experience showed, that already with proton beam intensities as low as 10 µA
a leak at a vacuum seal may occur after overheating it for only a few seconds.
- Program B: see Fig. 4B (11 kB)
Because of the scattering of protons passing through target material, the proton beam
spill measured with ionisation chambers down-stream from the target is proportional to the proton beam
intensity as long as the proton beam remains on axis. Therefore, the quotient of the measured IC currents
(I) divided by the actual proton beam current (I0) remains constant over the whole range of beam intensities
above 50-100 µA ( see also Fig. 5 {14 kB}).
This fact is exploited by the programmed electronics in comparing this ratio with individually set upper
and lower limits and triggering an interlock in case of out-of-limit values. Additionally, the hardware
limit mentioned above is also supported by this program.
At the moment, only the LOCAM2-units of the proton beam line sections behind Target E are
equipped with program B. It is planned to investigate, if also the section between Target M and E may be equipped
with program B. ( implemented in SD 2005).
This would improve the usefulness of the ionisation chambers at low intensities, which is quite
important for protecting the vacuum chambers of Target E and just in front of it with more
redundancy (see also Fig. 1 and 10) .
3. Monitoring the proton beam halo at collimators and slits.
Behind Target E and in front of the SINQ-target several beam-halo monitors are installed. They
consist of 2 or 4 segments of thin sheet-metal (0.1 mm Nickel) mounted with insulators in front
of the slits or collimators. If protons are hitting the copper of the slits or collimators, then
while they pass through one of the segments and with an efficiency of about 5 % they produce a current
flowing to the measuring device (LOGCAM2). These currents are processed with program B described
for the ionisation chambers. Additionally, a too high left-right or up-down asymmetry also
produces an interlock. At the operator's console the actual currents collected at the
different segments of the 8 halo monitors may be displayed with a repetition rate of 1 Hz
(see Fig. 6 {11 kB}). This display is
an important tuning tool for optimizing the passage of the megawatt proton beam through the Target station E
and for adjusting the proton beam centring at the SINQ-target.
4. Monitoring the proton beam transmission at critical locations.
There are 4 location along the 590 MeV high intensity megawatt proton beam line, where the proton beam current transmissions
are monitored:
location: |
expression to evaluate: |
HE-beam splitter (EHT) |
I(MHC1) - I(MHC2) - I(MBC1) |
Thin target station (TM) |
I(MHC3) - I(MHC4) - Losses @TM |
Thick target station (TE) |
I(MHC4) - I(MHC5) - Losses @TE |
Drift-tube (leading to SINQ) |
I(MHC5) - I(MHC6) |
The proton beam currents are measured with the monitors (50 MHZ high-frequency cavities) MHC1 to MHC6
and MBC1 (measures the proton beam current of the peeled-off protons for PIREX), which have only an accuracy of around 1 %
and have also to be re-calibrated (done manually by operators, which is considered by experts
as a main security risk) from time to time. The four transmissions are computed with
local processors with a repetition rate of 200 Hz. Because of the limited accuracy of the proton beam
intensity measurements the allowed losses are dependent on the proton beam currents. At Target E (TE)
e.g. the permitted width of the window is ±5µA near 0 µA and ±90µA at 2 mA proton beam current
(see Fig. 7 {7 kB}). In order to reduce
the amount of spurious interlocks produced by proton beam current fluctuations, a proton beam current dependent time constant for the
integration of the measured current values is applied. This time constant varies between
110 ms for 0 µA and 10 ms for proton beam currents larger than 1.5 mA
(see Fig. 8 {8 kB}). The losses
at TM are 1.6 % over the whole range of proton beam currents, whereas for the Target E (length = 4 cm graphite)
the losses are 28 % + 1.3 % per mA proton beam current (i.e. 30% at 1.8 mA).
The most important motivation for introducing the transmission monitoring at Target E was to
prevent the possibility of too much proton beam bypassing the graphite target material (rotating wheel of 6 mm width).
This non-scattered proton beam passes through the high intensity proton beam line to SINQ with a higher momentum and generates
a considerably smaller spot at the SINQ-target, which may be harmful for it (e.g. liquid metal target
for the MEGAPIE experiment).
Fig. 9 {10 kB} shows a Monte-Carlo simulation
(with the program TURTLE) of the 2 proton beam spots (scattered and non-scattered proton beam) at the location of the SINQ-target.
In order to have more redundancy for protecting the MEGAPIE-target
from being hit by too much of the narrow non-scattered proton beam, an
additional method has been presented in the PSI Large Research Facilities Scientific
and Technical Report 2001, Volume VI, p.34-35. The proposed slit will be put in place during the
shutdown 2004 and its usefulness tested during the HE-beam period 2004.
In order to see first
results please click here or have a look at the
article First beam tests with the new slit
collimator in the high intensity proton beam line to SINQ of the PSI Large Research Facilities Scientific
and Technical Report 2004, Volume VI, p.23-26.
5. Controlling correlated magnets in front of Target E with a super-knob device.
The magnetic fringe field of the (backwards) extraction magnet AHSW41 of the
PiE5 secondary
beam line (starting at Target E) is also deflecting the megawatt proton beam in front of the Target E.
In order to compensate this effect, 2 additional bending magnets named AHU and AHV are required
( see Fig. 10 {21 kB}). In this figure, the 2 red curves show
the central trajectories of the megawatt proton beam (coming from the left) for 120 MeV/c µ±
beams being extracted into the PiE5 beam line. In order to hit the Target E at the centre and keeping
the megawatt proton beam parallel to the axis, the current settings for the 2 compensation magnets AHU
and AHV have to be chosen for the different AHSW-currents (proportional to the momentum of the
extracted muons with 3.529 A/MeV/c) from the curves shown in Fig. 11 (7 kB).
To avoid wrong settings of these 3 magnets, which could lead very easily to a hole in one
of the nearby vacuum chambers, (see Fig. 10 and assume e.g. the current of the magnet AHV has the wrong sign)
their values cannot be set directly. Instead a super-combi device is used, which gets the
muon-momentum (in MeV/c) as input AHINP and transforms it with the help of a lookup table
(corresponding to Fig. 11) into magnet set-point values and then performs the magnet settings. Two
additional super-combi input parameters allow it similarly
with AHPOS to shift the proton beam horizontally at Target E parallel to the axis within the range of ±5 mm and/or
with AHWIN to vary the horizontal direction of the megawatt proton beam at Target E within the range of ±5 mr.
Thus, as long as this super-combi device works properly, there is no chance, that the proton beam can hit a wall
of a vacuum chamber near Target E. Between Target M and Target E there are also 2 horizontally
and 2 vertically acting steering magnets with a maximum deviation power of ±5 mr
(see Fig. 12 {27 kB}). Comparing
the drawn (in red) maximum possible (5 mr) proton beam centroid shifts reachable by these steering
magnets, it is also obvious, that no vacuum chamber walls (see Fig. 10) can be hit
with this 'worst-case' megawatt proton beam at regions where its spot size is small.
In the near future it is planned to add a second identical (with the exception of disabled set-point channels)
super-combi device to the existing one. This shadowing feature would certainly increase the safety, because if one of
the 2 units is malfunctioning, the other would still be able e.g. to check the back-readings of the 3 magnets and
generate an interlock in case of a magnet power-supply drop-out.
( implemented in SD 2004).

Last updated by
Urs Rohrer on 20-Feb-2006
|